Exploitation of KATP channels for cardiac surgery
Abstract
The many ways in which ATP-sensitive potassium (KATP) channels can be exploited for human benefit have expanded over recent decades. Especially since the early 2000s, research has improved our understanding of the components and mechanisms of KATP channels. They have the potential to have a prominent role in cardiac surgery. Pharmacologic and non-pharmacologic activation of KATP channels has been shown to be both cardioprotective and neuroprotective in early basic science and clinical studies. However, many questions remain unanswered and require further study, necessitating further basic science work and large human clinical trials. This review discusses the history and recent progress in the research relating to the use of KATP channels for cardiac surgery.
Keywords
INTRODUCTION
ATP-sensitive potassium (KATP) channels are present in many different tissues in humans, including cardiac muscle[1], vascular smooth muscle[2,3], lymphatics[4,5], liver[6,7], and pancreas[8,9]. These channels are clinically important because malfunction can lead to a variety of pathologies[10], and because they are pharmacologic targets of therapeutics[11,12]. Agents targeting these channels are already used to treat and even cure some diseases (neonatal diabetes and congenital hyperinsulinism are effectively treated with KATP channel inhibitors and KATP channel openers, respectively)[13,14], yet there is potential for further exploitation of KATP channels to benefit patients[15]. The goal of studying these channels is to gain information that will allow the development of new pharmacologic agents aimed at improving cardiac and neurologic outcomes after cardiac surgery.
Pathology associated with KATP channels can result from decreased or increased function, depending on the specific mutation and subunit affected. Loss of function of KATP channels results in hyperinsulinism and vascular hypercontractility[16,17], whereas gain of function leads to prolonged PR intervals, arrhythmias, and hypotension[2,3,18-20]. The pathology of Cantu syndrome (hypertrichosis-osteochondrodysplasia-cardiomegaly syndrome) results from genetic gain of function of KATP channel subunits, resulting in abnormalities including cardiac enlargement and ventricular hypertrophy, pericardial effusion, pulmonary hypertension, and hypertrichosis[11,21]. Understanding the abnormalities in Cantu syndrome is informative with regard to the non-pathologic role of KATP channels in human physiology[22]. Some pharmacologic agents that activate KATP channels may cause some of the same changes as those seen in Cantu syndrome, and this modulation of these channels has the potential to be beneficial[11,23,24].
The body of literature on the nature and role of KATP channels in various tissues has grown steadily over recent decades. A subset of literature focuses on the role of these channels in cardiac disease[25]. Activation of KATP channels is involved in cardioprotection or preservation of the myocardium during stress, such as the global ischemia imposed during cardiac surgery[26]. The channels are similarly involved in the protection of the neural cortex and spinal cord[27-29]. Previous authors have reviewed the early basic science of KATP channels[30-33]. In this review, we highlight recent evidence with a focus on the role of these channels in cardioprotection and neuroprotection during cardiac surgery.
STRUCTURE AND FUNCTION OF KATP CHANNELS
Cardiac myocytes contain two KATP channel entities: one located in the sarcolemmal membrane (sKATP) and a proposed mitochondrial channel (mitoKATP). The structure and function of sKATP and mitoKATP appear to be related but with important differences[34-36]. The well-characterized sKATP channel has been cloned and is present at a very high density in the heart (2,000-3,000/myocyte)[37]. The sKATP channel is a hetero-octamer of four pore-forming polypeptides of the inwardly rectifying K+ channel family Kir6.x and four sulfonylurea receptor subunits of the superfamily of ATP-binding cassette transporters[38-40]. The sulfonylurea receptor (SUR) subunit represents the site for blockade by sulfonylureas and stimulation by potassium channel openers and adenosine diphosphate (ADP). The Kir6.x subunit is the location for inhibition by ATP[38,40].
The mitoKATP channel may be primarily responsible for the cardioprotection provided by KATP channel modulation[26,41], but the sKATP channel is better characterized and may also be involved[42]. The physiological role of the sKATP channel in cardiac tissue involves modulating cellular function in response to stress such as metabolic inhibition[25,43]. Specifically, the channel is opened in response to stress and closed (inhibited) in the presence of ATP[44]. The function of the mitoKATP channel appears to involve organelle response to stress, with effects on both the volume and the function of the mitochondria[45-47].
The focus of this review is the role function of KATP channels in the myocardium. However, KATP channels are present in cardiac muscle, skeletal muscle, and smooth muscle, and their function in smooth muscle is worth noting in this discussion about the cardiovascular effects of KATP channels[48]. Diazoxide is a KATP channel opener that has been found to cause substantial arterial and arteriolar dilatation[49,50]. This agent was first used clinically as an antihypertensive agent before its potential as a cardioprotective agent was identified[51]. The side effects of diazoxide have limited its clinical use for hypertension, though it is still used clinically primarily to treat hypoglycemia[52-55]. The role of KATP channels in causing hypotension is primarily attributed to the vascular smooth muscle cells[2,3]; however, these channels are also found in vascular endothelial cells, and the action in endothelial cells may contribute to the effects on vascular tone[56,57].
Some progress has been made in understanding the molecular identity of the mitoKATP channel[45], but the quest to definitively characterize these channels continues. One of the challenges that has limited progress towards better understanding mitoKATP channels has been that KATP channel openers and blockers are both nonspecific; therefore, it is difficult to differentiate effects on sarcolemmal versus mitochondrial channels[24,58]. The determination of the implicated subunits and the structural identification of a mitoKATP channel would allow the prevention of undesirable side effects of nonspecific channel openers and inhibitors and allow for directed cardiac targeting for clinical use. By utilizing the genetic deletion of known sarcolemmal KATP channel subunits, it may be possible to indirectly identify subunits of a mitochondrial KATP channel involved in cardioprotection or neuroprotection[41,45,59].
Novel mitochondrial proteins encoded by CCDC51 and ABCB8 genes, with unknown function, have been implicated as proteins involved in a mitoKATP channel[45]. Known potential sarcolemmal KATP channel subunits (Kir6.1, Kir6.2, Kir1.1, SUR1) and non-KATP channel proteins (ROMK) have been systematically evaluated using pharmacologic blockade, genetic deletion, or genetic alteration (gain of function) in multiple mouse models to identify potential mitoKATP channel proteins involved in diazoxide cardioprotection[41,59-66]. However, no subunit has thus far been definitively implicated in cardioprotection by diazoxide. The characterization of the specific molecular profile of mitoKATP channels is a challenge that requires further research[36,67].
KATP CHANNELS AND CARDIOPROTECTION
KATP channels provide endogenous myocardial protection via coupling of cell membrane potential to myocardial metabolism, since these channels are inhibited by ATP and open during times of metabolic stress[44]. Pharmacologic opening of KATP channels mimics ischemic preconditioning (IPC) in multiple animal models[68-72]. Pharmacologic or non-pharmacologic opening of KATP channels with diazoxide affords protection to isolated myocytes when the channels are opened before the onset of stress, but not if administered after the onset of stress[73]. In an isolated heart model, opening KATP channels before ischemia and during early ischemia but not upon reperfusion facilitated cardioprotection[74]. The requirement that these channels are targeted prior to stress is critical because it limits the usefulness of targeting these channels for cardioprotection to situations such as cardiac surgery where a known subsequent ischemic insult occurs[75]. Diazoxide given throughout an ischemic episode led to maximal protection in human atrial trabeculae, suggesting that diazoxide could be a helpful additive to cardioplegia[76].
Cardiac surgery is necessary to treat various cardiac diseases, but the surgery paradoxically causes injury to myocardium that is often already compromised. Contributors to myocardial injury during cardiac surgery include exposure to hypothermic hyperkalemic cardioplegia[77], an imposed global ischemic period, and an already compromised myocardium. The clinical manifestation of the myocardial injury after cardiac surgery is myocardial stunning (MS). Myocardial stunning is defined as myocardial dysfunction despite the resumption of blood flow[78], similar to the clinical consequences following global ischemia for cardiac surgery when flow is restored. MS is defined clinically as the need for inotropic support after surgery for
Early studies in canines demonstrated reduced myocardial function after brief myocardial ischemia[80], indicating that patients undergoing cardiac ischemia required for heart surgery would have a decrease in myocardial function following reperfusion. The search for methods to protect the myocardium from such insults has been ongoing since cardiac surgery began in the 1950s. Many pharmacologic and nonpharmacologic methods have been identified with varying degrees of efficacy and clinical potential[81].The use of carefully designed cardioplegia solutions to facilitate cardiac arrest during surgery has been the primary method of protection from global ischemia, but the cardioplegia itself contributes to myocyte injury[82]. The use of KATP channel openers may offer protection against such injury. Advancements in understanding how myocardial KATP channels are involved in cardioprotection have occurred over the last several decades. [Table 1] This research has progressed towards safely using KATP channel openers for the benefit of human patients with cardiac disease, though they are not yet used clinically for this purpose.
Published research demonstrating or relating to the cardioprotective effects of KATP channel openers
Reference (author, year) | Model | Findings |
Noma, 1983[1] | Mammal myocytes | The KATP channel appeared important for regulation of cellular energy metabolism in cardiac cells |
Murry et al., 1986[83] | Canine model | Intermittent episodes of ischemia protected the myocardium by delaying cell death when the myocardium was later subjected to ischemia |
Grover et al., 1990[71] | Rat heart, Langendorff; canine model | Nicorandil resulted in improved contractility via indirect action, but cromakalim was directly cardioprotective |
Tseng et al., 1990[37] | Canine myocytes | Pinacidil increased efflux through KATP channels, and this was modulated by enzymatic reaction |
Gross et al., 1992[84] | Canine model | Benefits of preconditioning in dogs were abolished by blocking the KATP channel |
Auchampach et al., 1992[68] | Canine model | Blocking the KATP channel prevented benefits of IPC on infarct size after prolonged coronary occlusion |
Galiñanes et al., 1992[70] | Rat heart, Langendorff | The KATP channel opener lemakalim had anti-ischemic effects, but not in combination with high K+ cardioplegia |
Shigematsu et al., 1995[69] | Guinea pig ventricles | Ischemia causes activation of KATP channels, which contributes of recovery of contraction after reperfusion |
Inagaki et al., 1996[38] | Isolated proteins from rat and mouse | A family of SUR receptors determines the function of KATP channels |
Lawton et al., 1996[93] | Rabbit heart, Langendorff | Pinacidil provided better postischemic recovery compared with controls |
Lawton et al., 1996[92] | Rabbit heart, Langendorff | Pinacidil and aprikalim are comparable to St. Thomas’ solution for cardioprotection |
Garlid et al., 1997[26] | Rat hearts, Langendorff | Diazoxide was significantly more potent than sarcolemmal KATP at opening mitoKATP, implicating a role for mitoKATP in cardioprotection |
Shyng et al., 1997[40] | Genetically modified cells | The KATP channel pore is octameric or tetradimeric in structure. Each includes four Kir6.2 subunits and SUR1 subunits |
Lawton et al., 1997[94] | Rabbit heart, Langendorff | Pinacidil was cardioprotective, and this cardioprotection was lost with a KATP blocker |
Lawton et al., 1998[90] | Rabbit heart, Langendorff | Pinacidil was comparative to warm blood cardioplegia for systolic recovery |
Nakai et al., 2001[101] | Rabbit heart, Langendorff | Diazoxide and IPC were both cardioprotective. Effects were lost with a mitoKATP channel blocker |
Forbes et al., 2001[114] | Rat heart, Langendorff | Adding either diazoxide or pinacidil caused increased ROS, and this is blocked when 5-hydroxydecanoate or antioxidant is added |
Murata et al., 2001[104] | Rabbit myocytes | Attenuation of mitochondrial Ca2+ overload, because of partial mitochondrial depolarization by mitoKATP channels, provided cardioprotection |
Tsuchida et al., 2001[74] | Rabbit heart, Langendorff | Opening KATP channels before ischemia and during early ischemia, but not upon reperfusion, was important for cardioprotection |
Carroll et al., 2001[115] | Human atrial myocytes | Diazoxide causes preconditioning via mitochondrial swelling and free radical production |
Ockaili et al., 2001[121] | Rabbit model | 3-nitropropionic acid, a mitochondrial SDH inhibitor, has anti-ischemic effects due to mitoKATP channel opening |
McCully et al., 2002[131] | Swine model | Adding diazoxide to cardioplegia decreased myocardial apoptosis and mitochondrial damage |
Krenz et al., 2002[112] | Rat vascular smooth muscle cells | KATP channel opening by diazoxide or pinacidil led to ROS production from mitochondria |
Hanley et al., 2002[119] | Swine heart mitochondria | Succinate oxidation and SDH activity were inhibited by diazoxide, suggesting a cardioprotective mechanism other than KATP channel activation |
Wang et al., 2003[137] | Humans | Preconditioning with diazoxide was protective compared to cardioplegia alone, resulting in better hemodynamic recovery |
Suzuki et al., 2003[109] | Mouse heart, Langendorff | Diazoxide enhanced actional potential shortening during ischemia by activating sarcolemmal KATP channels |
Oldenburg et al., 2003[116] | Mouse heart proteins | The KATP channel opener P1075 opened mitoKATP channels and was cardioprotective via this action |
Dzeja et al., 2003[120] | Rat heart mitochondria; rat heart, Langendorff | Production of ROS and degradation of nucleotides were reduced by diazoxide, resulting in cellular protection |
Uchiyama et al., 2003[127] | Rat heart, Langendorff | Pharmacological preconditioning involved protein kinase C, and this involves adenosine, mitoKATP activation, and nitric oxide |
Rousou et al., 2004[100] | Rabbit heart, Langendorff | Diazoxide added to cardioplegia was cardioprotective via preservation of mitochondrial physiology |
Wakahara et al., 2004[86] | Rat heart, Langendorff | The mitoKATP channel blocker and antioxidant abolished postischemic recovery of contractile function by diazoxide but not by IPC |
Ardehali et al., 2004[111] | Rat liver mitochondria | The mitoKATP channel composition includes SDH as a component |
Eaton et al., 2005[125] | Rat heart, Langendorff | IPC and diazoxide appeared to have protective effects via a mechanism involving reactive oxygen species generation before ischemia onset |
Mizutani et al., 2005[77] | Rabbit myocytes | Cellular swelling was detrimental for cardiac contractility, and diazoxide attenuated swelling |
Busija et al., 2005[122] | Piglet mitochondria | ROS production was increased by diazoxide, and this was likely via inhibition of SDH |
Deja et al., 2006[76] | Human atrial trabeculae | Diazoxide given throughout an ischemic episode led to maximal protection, suggesting diazoxide could be a helpful additive to cardioplegia |
Yonemochi et al., 2006[130] | Rat myocytes | The mitoKATP channels acted as a trigger and mediator of cardioprotection through ΔΨm loss |
Prasad et al., 2006[61] | Mouse heart, Langendorff | The sarcolemmal KATP channel appeared necessary for exaggerated swelling and reduced contractility after cardioplegia |
Mizutani et al., 2006[82] | Rabbit myocytes | Diazoxide abolished the swelling and reduced contractility caused by St. Thomas’ cardioplegia |
Kim et al., 2006[128] | Rat myocytes | Diazoxide cardioprotection appeared to occur via protein kinase C pathway |
Al-Dadah et al., 2007[106] | Rabbit myocytes | The attenuation of both swelling and reduced contractility by diazoxide was unchanged by adding KATP blockers |
Flagg et al., 2008[44] | Mouse myocytes | Atrial and ventricular KATP channels have fundamentally different structures, including a difference in SUR1 |
Wojtovich et al., 2008[110] | Rat heart, Langendorff; rat mitochondria | MitoKATP activation in IPC may involve complex II inhibition by malonate |
Deja et al, 2009[138] | Humans | Adding diazoxide to cardioplegia improved myocardial protection |
Sellitto et al., 2010[41] | Mouse myocytes | Diazoxide did not open the ventricular sarcolemmal KATP channel. The mechanism involved a pathway involving SUR1 |
Zhang et al., 2011[108] | Mouse myocytes | HMR 1098, a KATP channel blocker, is nonspecific. Methods such as genetic deletion are needed to confirm channel subunit involvement in cardioprotection |
Maffit et al., 2012[107] | Human myocytes | Diazoxide lessened swelling with or without inhibition of KATP channel |
Anastacio et al., 2013[60] | Mouse mitochondria | The benefit of diazoxide during stress involves inhibition of SDH and possibly opening of mitoKATP |
Anastacio et al., 2013[103] | Mouse mitochondria | Inhibition of mitoKATP channels with 5-HD reduced mitochondrial volume during stress, indicating a role for mitoKATP channel with diazoxide |
Anastacio et al., 2013[124] | Mouse mitochondria | The cardioprotection of diazoxide was independent of the SUR1 subunit of the KATP channel, though it may occur via inhibition of the SDH enzyme complex |
Garlid et al., 2013[117] | Rat heart mitochondria | The mitochondrial ROS involved in cardioprotection by IPC and diazoxide appeared to be hydroxyl radical (HO·) |
Janjua et al., 2014[73] | Mouse ventricular myocytes, human atrial myocytes | Diazoxide was only cardioprotective if administered at the onset of stress and not if administered after onset of stress |
Henn et al., 2015[62] | Mouse ventricular mitochondria | The subunits Kir1.1, Kir3.1, and Kir3.4 all could possibly be involved in cardioprotection caused by diazoxide |
Henn et al., 2015[63] | Mouse myocytes | The Kir6.1 subunit improved myocyte tolerance to stress, but the mechanism was unknown |
Henn et al., 2016[65] | Mouse myocytes | The negative effects of cardioplegia were decreased in Kir6.1GOF myocytes |
Makepeace et al., 2018[134] | Mouse heart, Langendorff | Adding diazoxide to cardioplegia provided improved recovery after ischemia |
Paggio et al., 2019[45] | HeLa cells with plasmids encoding KATP proteins and mouse models | Over- or under-expression of mitoKATP is detrimental. Cardioprotection by diazoxide is lost when mitoKATP is suppressed |
Suarez-Pierre et al., 2020[135] | Swine model | Diazoxide preserved systolic and diastolic ventricular function after ischemia in a large animal model |
Ahmad et al., 2021[126] | Mouse myocytes | S-nitrosating agent and diazoxide are cardioprotective individually, but the beneficial effect was lost when they were combined |
Velez et al., 2022[136] | Swine model | Diazoxide reduced myocardial stunning and facilitated separation from cardiopulmonary bypass |
Wang et al., 2023[59] | Mouse myocytes and hearts | Neither Kir1.1 (ROMK) nor SUR1 were involved in the mechanism of cardioprotection by diazoxide |
In 1983, Noma demonstrated that the KATP channel was involved in the regulation of energy metabolism in cardiac cells[1]. For the past 40 years since Noma’s study was published, there have been ongoing efforts to understand and utilize KATP channels for patients with cardiac pathology. Murry et al. published data demonstrating non-pharmacologic cardioprotection with IPC in dogs, which was a catalyst for subsequent work exploring the mechanism of IPC[83]. In 1992, Gross et al. demonstrated that the benefits of preconditioning in dogs were abolished by blocking the KATP channel pharmacologically[84,85]. The link between cardioprotection and KATP channels was then established, and led to further studies seeking to exploit the KATP channel for pharmacologic cardioprotection[86].
In the mid- to late-1990s, many experiments were performed on isolated animal hearts in a Langendorff apparatus model to further explore the potential of pharmacologic cardioprotection with different KATP channel openers. The Langendorff perfused heart model refers to a procedure that is useful for experiments in which the goal is to evaluate heart function in response to medications or ischemia-reperfusion. The method involves using an isolated heart perfused via the aorta and, thus, the coronary arteries[87-89]. Such models can utilize physiologic crystalloid perfusion or blood perfusion to more closely mimic clinical situations[90]. Pinacidil and aprikalim are two KATP channel openers that were used in early studies to assess cardioprotection, and both were found to be protective during cardiac ischemia. The benefit of pinacidil was compared to St. Thomas’ solution (a commonly used hyperkalemic cardioplegia solution[91]); Pinacidil provided better postischemic recovery in isolated hearts after ischemia[90,92,93]. KATP channel openers were found to be effective in crystalloid hypothermic, hyperkalemic cardioplegic solutions, in blood cardioplegia, and in the acutely injured heart[90,93,94]. The cardioprotective effect of pinacidil when added to hypothermic depolarizing cardioplegia was lost with a KATP blocker, suggesting that the benefit involved KATP channels[94].
Multiple KATP channel openers have been studied for cardioprotection, and each has a different pharmacology. Pinacidil was used in many early studies evaluating KATP channel openers and cardioprotection, but many researchers have since focused on diazoxide due to its proposed specificity for mitoKATP channels, unlike most other KATP channel openers[34]. Garlid found that diazoxide has a 2,000-fold greater affinity for mitoKATP compared to the sarcolemmal KATP channel[95]. However, some of the other potassium channel openers including cromakalim, nicorandil, and pinacidil may still have some role in some role in cardioprotection[96]. Comparisons of cromakalim and diazoxide suggested that diazoxide has less effect on cardiac action potential duration than some other potassium channel openers[97]. The shortening of the cardiac action potential has been proposed as a mechanism of cardioprotection by KATP channel openers[98].
THE MOLECULAR MECHANISM OF CARDIOPROTECTION AFFORDED BY KATP CHANNEL ACTIVATION IS UNKNOWN
The understanding of KATP channels on mitochondrial membranes developed in the 1990s after they were characterized by Inoue et al. in 1991[99]. The interest in the mitoKATP channel for cardioprotection was proposed to be based on responses of mitochondria to stress. As previously stated, mitoKATP channels open during stress, which facilitates increased mitochondrial volume and reduced calcium overload, both of which are beneficial for mitochondrial function and overall cellular adaptation[45,100].
Diazoxide and perhaps other KATP channel openers were initially suggested to be cardioprotective via a mechanism involving a mitoKATP channel rather than a sKATP channel in the few years after the mitoKATP channels were characterized[26,33]. Efforts to define the role of both sarcolemmal and mitochondrial channels involved in cardioprotection were begun. Pharmacologic cardioprotection and non-pharmacologic cardioprotection using IPC were compared: both were cardioprotective, and the cardioprotection initially appeared to be inhibited in the presence of a selective mitoKATP channel blocker, 5-hydroxydecanoate[101]. Additionally, when a mitoKATP channel blocker was added to isolated hearts with either diazoxide or IPC, this abolished the improvement in contractility after ischemia that had been afforded by diazoxide but not by IPC, suggesting that a mitoKATP was critical for diazoxide’s mechanism but not for IPC[86]. In line with these findings, a later study using genetic deletion found that diazoxide’s cardioprotection was not due to action at a sKATP channel[41].
Ultimately, the molecular mechanism of action at a proposed mitoKATP channel remains unknown. Potential mechanisms largely focus on the mitochondria and include KATP channel-related and non-related mechanisms[42]. It is also possible that the effects of diazoxide and other KATP channel openers can be attributed to a combination of mechanisms[102].
POTENTIAL MECHANISMS OF CARDIOPROTECTION (KATP CHANNEL AND CHANNEL-INDEPENDENT)
Mitochondrial and cellular volume alteration
Both cellular and mitochondrial volume alterations have been investigated in the search for mechanisms of cardioprotection relating to KATP channels and their openers. While opening sKATP channels results in K+ efflux from the cell[30], opening of a mitoKATP channel results in K+ influx from the cytosol into the mitochondria and mitochondrial swelling, suggesting a potential basis for mitoKATP-dependent changes in mitochondrial and cellular volume alteration during to stress[32,46,67]. Interestingly, opening the mitoKATP channel was associated with changes in mitochondrial matrix volume, calcium concentration, and respiration[100]. Diazoxide facilitated mitochondrial swelling in ischemia[103] (whereas mitochondria otherwise tend to contract in this setting) and reduced mitochondrial calcium accumulation, which are beneficial for mitochondrial function[100]. In animal myocytes undergoing ischemia and reperfusion, pharmacologic opening of mitoKATP channels was associated with decreased matrix calcium overload because of mitochondrial membrane depolarization[104].
The mechanisms and effects of cellular swelling observed in ischemic myocytes secondary to stress have been studied for several decades[105], and the prevention of swelling has been suggested to be a potential mechanism of KATP cardioprotection. Studies in the 2010s elucidated relationships between cellular volume changes during stress and myocyte contractility. Stresses that caused cellular swelling (hypoosmotic stress; exposure to hyperkalemic, hypothermic cardioplegia; or exposure to metabolic inhibition) resulted in reduced contractility that was prevented with the addition of KATP channel opener diazoxide[77,82,106]. Hyperosmotic stress resulted in myocyte shrinkage and improved contractility, and this inverse relationship between function and myocyte size suggested a cellular mechanism for myocardial stunning[77]. Thus, a proposed cellular model of myocyte stunning was created to test mechanisms of diazoxide cardioprotection[77].
Interestingly, in myocytes, an open sKATP channel appeared necessary for the observed cellular swelling and reduced contractility after exposure to hypothermic, hyperkalemic cardioplegia[61]. An interplay between cellular volume and mitochondrial volume may be important in cardioprotection afforded by KATP channel openers.
Pharmacologic channel blockers have also been utilized to determine potential mechanisms of cardioprotection and implicated channel subunits. The attenuation of both swelling and reduced contractility by diazoxide was unchanged by sarcolemmal or mitochondrial KATP channel blockers, contradicting other work with channel blockers[106-108]. This led to the proposal that pharmacologic channel blockers are nonspecific and only definitive methods such as genetic deletion could confirm channel subunit involvement in cardioprotection.
While the mitoKATP channel became a focus of cardioprotection, there was still evidence suggesting a role for the sKATP channel. A study using the Langendorff model found that diazoxide’s cardioprotection was provided via sKATP channels rather than mitoKATP channels[109]. However, work using whole-cell voltage clamping of ventricular myocytes determined that diazoxide does not open sarcolemmal KATP channels; this led the investigation of diazoxide cardioprotection away from the sKATP channel[41].
Mitochondrial respiration
There are several potential KATP-independent mechanisms of diazoxide cardioprotection at the mitochondrial level including the inhibition of succinate dehydrogenase (SDH), increased reactive oxygen species signaling, and protein kinase C activation[42,110-117]. While the majority of studies have implicated KATP channels, some have emphasized that diazoxide and other openers have important KATP channel-independent actions, and therefore cardioprotective mechanisms remain unclear[42,118].
KATP channel opener diazoxide is a known inhibitor of mitochondrial enzyme complex II, SDH, a component of the electron transport chain[119,120]. Consistent with this idea, other SDH inhibitors have been found to be cardioprotective, and reversal of SDH and succinate accumulation is the primary driver of mitochondrial reactive oxygen species (ROS) production that underlies ischemia/reperfusion injury[110,121-123]. The exact nature of the interaction between SDH and mitoKATP is unknown. In myocyte experiments, diazoxide cardioprotection required inhibition of SDH, and the role of KATP channel activity was not clear[60]. Diazoxide inhibited SDH in the presence of mitoKATP channel inhibitor 5-hydroxydecanoate, and SDH inhibition alone did not lead to an increase in mitochondrial volume (a surrogate for mitoKATP activity)[124]. These findings suggested that SDH is not directly upstream of a mitoKATP channel.
Reactive oxygen species mimic IPC (attributed to activity at a KATP channel) and antioxidants block IPC[114,115]. The role of ROS was investigated as a potential cardioprotective mechanism of diazoxide[67]. Diazoxide and pinacidil increased ROS in cardiomyocytes, and this increase was blocked with the co-administration of 5-hydrodecanoate or an antioxidant, supporting the hypothesis that ROS are involved in cardioprotection facilitated by mitoKATP channels[114]. In isolated perfused rat hearts, ROS generation prior to ischemia onset contributed to the cardioprotection of both IPC and diazoxide[125]. In animal models, glutathione (an antioxidant) administered before ischemia prevented cardioprotection by diazoxide, via prevention of inhibition of SDH or the inhibition of ROS formation[60]. Similarly, a mitochondrial-targeted antioxidant that inhibits mitochondrial enzyme complex I MitoSNO (given at reperfusion) reduced cardioprotection by diazoxide, suggesting an interplay at the mitochondrial level [Figure 1][126]. Data published in 2019 provided further evidence that mitoKATP is important for redox homeostasis by showing that diazoxide results in increased ROS in wild-type mice, but not in cells lacking proposed mitoKATP channel subunits[45].
Figure 1. Electron transport chain with KATP channel and actions of MitoSNO and diazoxide. The schema simplifies the activity of the inner membrane of the mitochondrion, including the proposed KATP channel and electron transport chain. At the bottom of the figure, a photo taken by electron microscopy from the Lawton laboratory, of individual mitochondria. In the schema, diazoxide is depicted as having an inhibitory effect on Complex II (succinate dehydrogenase) while activating the KATP channel. The cardioprotection by diazoxide may occur due to either of the mechanisms or another mechanism. MitoSNO has an inhibitory effect on Complex I, which prevents SDH accumulation, and this is thought to be the cardioprotective mechanism of MitoSNO. While each is cardioprotective via these mechanisms, these two agents have a synergistic negative effect[126]. This figure is used with permission from Elsevier (obtained September 4, 2023, license number 5621920353581)[126]. MitoSNO: Mitochondria-targeted S-nitrosating agent; NADH: nicotinamide adenine dinucleotide, reduced form; NADþ: nicotinamide adenine dinucleotide, oxidized form; ROS: reactive oxygen species; ATP: adenosine triphosphate; ADP: adenosine diphosphate; Cyt C: cytochrome C.
Some have suggested that KATP cardioprotection and IPC involve the activation of protein kinase C (PKC) (specifically PKC-€) and may be blocked by PKC inhibition or genetic deletion[112,127,128]. Diazoxide has also been implicated in the translocation of PKC-€ from the cytosol to the mitochondria as a mechanism of cardioprotection[128].
Others have evaluated the role of apoptosis in cardioprotection via the exploitation of KATP channels. In a myocyte model, diazoxide and pinacidil protected rat ventricular myocytes against apoptosis[129]. Another myocyte study found that diazoxide was protective against apoptosis, although protection depended on the timing of treatment[130]. The cardioprotection associated with diazoxide in a swine model was found to result from decreased myocyte apoptosis and mitochondrial damage[131]. Recent reviews have also discussed the inhibition of apoptosis as a potential mechanism of KATP channel modulation in cardioprotection[132].
TRANSLATIONAL AND HUMAN STUDIES USING KATP CHANNEL OPENERS FOR CARDIOPROTECTION
The studies performed in the 2000s and early 2010s led to knowledge of the basic mechanisms of KATP channels within cells and organelles, providing the framework for understanding how KATP openers are beneficial for cardioprotection, elucidating their mechanisms of action. Over recent years, researchers have then turned to the potential role of these channels as pharmacologic targets in human patients. To facilitate the understanding of potassium channel openers at tissue and organism levels, isolated heart models and intact large animal models that mimic conditions of myocardial ischemia during cardiac surgery have been developed to test the systemic hemodynamic effects associated with KATP channel opener diazoxide.
An early study in 2005 comparing diazoxide to control in a porcine model found that diazoxide did not provide cardioprotection (infarct size and systolic function) after myocardial ischemia[133]. The authors acknowledged that their results were incongruent with others’ findings and postulated that this could be due to preconditioning effects of anesthetics or an incorrect dose of diazoxide. Recent studies have been more promising. In an isolated mouse heart model, adding diazoxide to cardioplegia led to improved diastolic function following a period of global ischemia[134].Two subsequent studies were conducted in swine models. In the first, swine treated with hypothermic, hyperkalemic cardioplegia with diazoxide (single dose) prior to a 2-h global ischemic period were found to have improved systolic and diastolic ventricular function compared to cardioplegia alone [Figure 2][135]. In the second, swine underwent 30 min of occlusion of the left anterior descending artery prior to 2 h of global ischemia protected with cardioplegia or cardioplegia with diazoxide (dosed every 20 min) [Figure 3][136]. Compared to cardioplegia alone, animals that received diazoxide had decreased myocardial stunning and shortened time to separate from cardiopulmonary bypass [Figure 4][136]. These studies provided some of the most convincing preclinical data to date that diazoxide will be beneficial as an additive to cardioplegia in humans undergoing cardiac surgery requiring global ischemia. It is important to acknowledge the limitations of the translational models that have been widely used to study KATP channels and cardioprotection. These models may not provide sufficient confidence to translate to human pathophysiology. These limitations highlight the importance of randomized clinical trials in humans before widespread adoption.
Figure 2. Summary of the benefits with use of KATP channel opener diazoxide in a translational swine model of hypothermic cardioplegic arrest and prolonged global ischemia similar to a prolonged global ischemia model for clinical cardiac surgery. Swine undergo cardiopulmonary bypass and prolonged global ischemia of the heart via placement of aortic cross clamp (120 min) with one dose of cardioplegia, similar to clinical cardiopulmonary bypass. Animals are randomized to one of two groups: cardioplegia alone or cardioplegia plus diazoxide. Animals who underwent cardioplegia plus diazoxide in this model had preserved systolic and diastolic function, suggesting that adding diazoxide to cardioplegia may result in improved outcomes[135]. This model simulates the clinical situation of a patient presenting to the operating room for a prolonged global ischemic period for cardiac surgery protected with one dose of cardioplegia[135]. This figure is used with permission from Elsevier (obtained September 4, 2023, license number 5621921258491)[135]. CPB: Cardiopulmonary bypass.
Figure 3. Photograph of porcine heart in the Lawton laboratory following cannulation for cardiopulmonary bypass, placement of left ventricular pressure catheter, and placement of left anterior descending tourniquet for regional ischemia prior to a longer global ischemia in a model simulating clinical cardiopulmonary bypass with an acutely injured heart. Regional ischemia is evident in this porcine heart after occlusion of the left anterior descending coronary artery for 30 min for regional ischemia. Subsequently, the heart will be exposed to two hours of global ischemia protected with cardioplegia with or without diazoxide, followed by reperfusion[136]. This model simulates the clinical situation of a patient presenting to the operating room with ongoing ischemia prior to a coronary revascularization procedure with prolonged global ischemia[136]. This figure is used with permission from Elsevier (obtained December 12, 2023, license number 5686380805849)[136].
Figure 4. KATP channel opener diazoxide was associated with improved left ventricular ejection fraction at 30 min after reperfusion in a porcine model of 30 min of regional ischemia followed by 2 h of global ischemia, simulating clinical cardiopulmonary bypass in the acutely injured heart prior to cardiac surgery. Using a translational porcine model of regional ischemia (30 min) followed by 2 h of global ischemia, animals were randomized to one of two groups: cardioplegia alone (red, n = 6) or cardioplegia plus diazoxide (blue,
Two small, randomized trials in humans have investigated the cardioprotective effects of diazoxide in humans[137,138]. Wang et al. (2003) randomized 40 patients undergoing coronary artery bypass grafting to receive either 1.5 mg/kg diazoxide infusion or placebo intravenously prior to undergoing global ischemia for cardiac surgery. They found improved hemodynamic recovery after surgery in patients who received diazoxide, though they noted that further studies were needed to determine an optimal dosing protocol[137]. Deja et al. (2009) randomized 40 patients to receive intermittent warm blood cardioplegia that was supplemented with 100 µmol/L diazoxide or placebo prior to global ischemia for cardiac surgery[138]. They found that patients treated with diazoxide required less inotropic support and had higher cardiac indices after global ischemia[138]. Both human studies were small, involved low-risk patients, and were primarily aimed at demonstrating feasibility and safety, which were achieved. Neither human study evaluated a KATP channel opener in hypothermic, hyperkalemic cardioplegia prior to global ischemia. This is an important next step, as normothermic, hyperkalemic cardioplegia (in contrast to hypothermic) has not been implicated in myocyte swelling and reduced contractility following stress[82]. Additional studies are needed with KATP channel openers in hypothermic, hyperkalemic cardioplegia to demonstrate feasibility and, subsequently, safety and efficacy prior to widespread human use.
THE ROLE OF KATP CHANNELS IN NEUROPROTECTION
KATP channels may also have a role in neuroprotection during cardiac surgery that involves deep hypothermic circulatory arrest for aortic replacement, congenital heart surgery, pulmonary endarterectomy, repair of thoracoabdominal aneurysms, or other complex procedures. The primary methods of neuroprotection for such surgery include: deep hypothermic circulatory arrest (DHCA), antegrade cerebral perfusion, and retrograde cerebral perfusion[139]. Similar to cardioprotection for cardiac surgery, methods of neuroprotection are imperfect. A recent study of patients undergoing aortic arch surgery with HCA and unilateral ACP had a 4.8% incidence of permanent neurological dysfunction[140]. A wide variety of pharmacologic agents have been studied to improve neurologic outcomes after cardiac surgery[141,142]. Among the agents that may be useful in these efforts is KATP channel opener diazoxide[27,143].
Several studies in the late 1990s explored the role of KATP channels in neuroprotection [Table 2]. KATP channel openers have been demonstrated to depolarize hippocampal mitochondria, the area of greatest injury in DHCA[144]. KATP channels were neuroprotective during hypoxia in rat neocortical tissues[145], and diazoxide preserved neuronal-vascular function after cerebral ischemia in a swine model[146]. Diazoxide was an effective form of pharmacologic preconditioning in canine models, as evidenced by both improved clinical neurologic scores and histopathology compared to controls[28,147]. Similarly, diazoxide pretreatment resulted in improved neurologic outcomes in a spinal cord injury model, and the suggested mechanism was KATP channel activity[29]. MitoKATP channel openers have also been demonstrated to inhibit apoptosis in neurons by preserving mitochondrial inner membrane potential[148].
Published research demonstrating or relating to the importance of the KATP channel in neuroprotection
Reference (author, year) | Model | Findings |
Garcia de Arriba et al., 1999[145] | Rat neocortical tissue | ATP-dependent potassium channels were neuroprotective during hypoxia in rat neocortical tissues |
Domoki et al., 1999[146] | Swine model | Diazoxide preserved neuronal-vascular function after cerebral ischemia in newborn pigs |
Debska et al., 2001[144] | Rat hippocampal tissue | Potassium channel openers depolarized hippocampal mitochondria |
Shake et al., 2001[28] | Canine model | Intravenous diazoxide was neuroprotective in dogs undergoing cardiopulmonary bypass |
Caparrelli et al., 2002[29] | Rabbit model | Diazoxide pretreatment resulted in improved neurologic outcomes, and the mechanism appeared to be due to the KATP channel activity |
Teshima et al., 2003[148] | Cerebellar neurons | MitoKATP channel openers inhibited apoptosis by preserving mitochondrial inner membrane potential |
Barreiro et al., 2006[147] | Canine model | Pretreatment with diazoxide plus HCA led to improved neurologic outcomes versus HCA alone |
Yamanaka et al., 2018[151] | Mouse model | Diazoxide and EPO were synergistically protective of the spinal cord after ischemia with upregulation of a common receptor |
Yamanaka et al., 2019[152] | Mouse model | Diazoxide and EPO were synergistically protective of the spinal cord after ischemia via upregulation of NGF |
Yamanaka et al., 2019[150] | Mouse model | Oral diazoxide preserved motor function in spinal cord ischemia-reperfusion injury by the STAT3 pathway |
Ikeno et al., 2023[153] | Mouse model | Direct and indirect activation of mitoKATP channels were involved pharmacological spinal cord protection with motor function preservation |
The mechanism of diazoxide for neuroprotection is similar to one of many proposed for its cardioprotective action. Diazoxide is a KATP channel opener that inhibits complex II and maintains mitochondrial membrane potential. This prevents mitochondrial dysfunction and cell death following DHCA and injury due to N-methyl-D-aspartate (NMDA) excitotoxicity via unique pathways and could be utilized to produce synergistic benefit with NMDA blockade during cardiac surgery with DHCA [Figure 5][149].
Figure 5. Mechanistic depiction of neuron mitochondria with ketamine, glutamate, diazoxide, and other second messengers and potential mechanisms of injury during hypothermic circulatory arrest. This picture depicts a neuron with intracellular, intramitochondrial, and nuclear potential mechanisms of injury during hypothermic circulatory arrest. Both ketamine and diazoxide have potential neuroprotective effects. Ketamine is depicted here as affecting the NMDA receptors and subsequent second messengers within cytosol. Diazoxide is depicted as acting on the mitochondrial membrane at the mitoKATP channel and Complex II. Both pathways eventually affect ROS within the mitochondria. Downstream effects of both pathways are also thought to involve mechanisms within the nucleus that lead to DNA changes and apoptosis. Exploration of positive synergism of these agents is one option for future directions in neuroprotection research[149]. This figure was created by Mary Ann Wilson, PhD, and is used with her permission. A version of this figure was also previously published[149]. This figure is used with permission from Elsevier (obtained December 7, 2023, license number 5683710387270). ROS: Reactive oxygen species; MitoKATP: mitochondrial KATP channel; AMPA:
Early studies on neuroprotection focused on the brain cortex, but more contemporary work evaluated KATP channels and protection of the spinal cord, which is applicable to cardiac surgical procedures on the descending aorta that have a known complication of paraplegia from spinal ischemia. Diazoxide attenuated spinal cord ischemia-reperfusion injury, and motor function after spinal cord ischemia was improved in a mouse model with diazoxide compared to controls, an action that appeared to be associated with expression of the signaling transducer and activator of transcription (STAT) 3 pathway[150]. These results have been confirmed by several others that have demonstrated the potential of diazoxide to reduce spinal cord injury following ischemia[151-153].
Further work is needed to characterize the mechanisms involved in neuroprotection provided by diazoxide and its potential use in cardiac surgery.
FUTURE DIRECTIONS
Future work will further advance the understanding of the function and potential of KATP channels for the benefit of patients undergoing cardiac surgery. Perhaps one of the most anticipated future endeavors is a large clinical trial using diazoxide as an additive to cardioplegia. While the use of diazoxide for cardioprotection in cardiac surgery patients has been demonstrated as safe and feasible[137,138], more data are needed in high-risk human patients and with the use of hypothermic, hyperkalemic blood cardioplegia.
Important fundamental questions remain about KATP channels. One unanswered question was made apparent in a recent study in which diazoxide was combined with a mitochondria-targeted S-nitrosating agent (mitoSNO). Each of these agents was known to be cardioprotective individually. However, the cardioprotective mechanism of diazoxide was found to be exclusive of the mechanism of MitoSNO; when combined, these agents were not beneficial[126]. The authors concluded that using nitric oxide donators could be detrimental in the presence of diazoxide, and this requires further investigation. The idea of exploring the positive and negative synergism of diazoxide with various other pharmacological agents should be further studied in both cardioprotection and neuroprotection. It will be critical to know which agents might enhance or negate the beneficial effects of diazoxide or other KATP agents.
Identification of the potential components of KATP channels and their roles in cardioprotection and neuroprotection, as well as non-channel mechanisms of action of pharmacologic channel openers, will require ongoing investigation. The latest work on the components of KATP channels in cardioprotection found that two specific subunits - ROMK and SUR1 - are not implicated in myocardial protection[59]. The subunits implicated in cardioprotection and neuroprotection remain to be determined. Answering this could lead to specific and targeted methods to exploit this channel pharmacologically to protect both the heart and the brain during cardiac surgery.
SUMMARY
This article reviews the background of KATP channels in the context of their potential use for myocardial protection and neuroprotection, summarizes recent data supporting their use, and outlines future directions for the role of these agents in cardiac surgery. Since it was first understood that KATP channels were present in cardiac cells and that they mimic IPC, research has advanced towards the goal of developing pharmacologic agents that could target these channels to improve outcomes for patients after cardiac surgery. Progress has been made using: genetic deletion to characterize the potential implicated protein subunits of the involved channels; pharmacological research and single cell voltage clamping to differentiate agents that result in channel activation; translational animal studies to demonstrate feasibility, safety, and potential benefit and small human randomized trials to demonstrate feasibility. Recently, efforts have focused specifically on a diazoxide because of its specificity for mitoKATP channels, and this agent shows promise for being able to facilitate cardioprotection and neuroprotection for patients. Continued investigation of KATP channels and their precise molecular mechanism of action will lead and support a broader understanding of the potential ways to target these channels to improve the lives of human patients.
DECLARATIONS
Acknowledgments
We thank Dr. Mary Ann Wilson for her contribution to Figure 5 and for her permission to use this figure.
Author’s contributions
Literature review, writing, and editing of the manuscript: Bradshaw A
Literature review, writing, and editing, the conceptualization and critical analysis of the review: Lawton JS
Availability of data and materials
Not applicable
Financial support and sponsorship
None.
Conflicts of interest
Both authors declared that there are no conflicts of interest.
Ethical approval and consent to participate
Not applicable
Consent for publication
Not applicable
Copyright
© The Author(s) 2023.
REFERENCES
2. Aziz Q, Thomas AM, Gomes J, et al. The ATP-sensitive potassium channel subunit, Kir6.1, in vascular smooth muscle plays a major role in blood pressure control vascular biology. Vasc Biol 2014;64:523-9.
3. Li A, Knutsen RH, Zhang H, et al. Hypotension due to Kir6.1 gain-of-function in vascular smooth muscle. J Am Heart Assoc 2013;2:e000365.
4. Davis MJ, Kim HJ, Nichols CG. KATP channels in lymphatic function. Am J Physiol Cell Physiol 2022;323:C1018-35.
5. Davis MJ, Castorena-Gonzalez JA, Kim HJ, Li M, Remedi M, Nichols CG. Lymphatic contractile dysfunction in mouse models of Cantú Syndrome with KATP channel gain-of-function. Function 2023;4:zqad017.
6. Alberici LC, Oliveira HC, Paim BA, et al. Mitochondrial ATP-sensitive K+ channels as redox signals to liver mitochondria in response to hypertriglyceridemia. Free Radic Biol Med 2009;47:1432-9.
7. Zhou M, Yoshikawa K, Akashi H, et al. Localization of ATP-sensitive K+ channel subunits in rat liver. World J Exp Med 2019;9:14-31.
8. McTaggart JS, Clark RH, Ashcroft FM. The role of the KATP channel in glucose homeostasis in health and disease: more than meets the islet. J Physiol 2010;588:3201-9.
9. Bennett K, James C, Hussain K. Pancreatic β-cell KATP channels: hypoglycaemia and hyperglycaemia. Rev Endocr Metab Disord 2010;11:157-63.
10. Olson TM, Terzic A. Human KATP channelopathies: diseases of metabolic homeostasis. Pflugers Arch 2010;460:295-306.
11. Nichols CG. Adenosine triphosphate-sensitive potassium currents in heart disease and cardioprotection. Card Electrophysiol Clin 2016;8:323-35.
12. Nichols CG. Personalized therapeutics for KATP-dependent pathologies. Annu Rev Pharmacol Toxicol 2023;63:541-63.
13. Brar PC, Heksch R, Cossen K, et al. Management and appropriate use of diazoxide in infants and children with hyperinsulinism. J Clin Endocrinol Metab 2020;105:3750-61.
14. Lang V, Light PE. The molecular mechanisms and pharmacotherapy of ATP-sensitive potassium channel gene mutations underlying neonatal diabetes. Pharmacogen Pers Med 2010;3:145-61.
15. Kharade SV, Nichols C, Denton JS. The shifting landscape of KATP channelopathies and the need for “sharper” therapeutics. Future Med Chem 2016;8:789-802.
16. Saint-Martin C, Arnoux JB, de Lonlay P, Bellanné-Chantelot C. KATP channel mutations in congenital hyperinsulinism. Semin Pediatr Surg 2011;20:18-22.
17. Gloyn AL, Pearson ER, Antcliff JF, et al. Activating mutations in the gene encoding the ATP-sensitive potassium-channel subunit Kir6.2 and permanent neonatal diabetes. N Engl J Med 2004;350:1838-49.
18. Antzelevitch C, Barajas-Martinez H. A gain-of-function I(K-ATP) mutation and its role in sudden cardiac death associated with J-wave syndromes. Heart Rhythm 2010;7:1472-4.
19. Levin MD, Zhang H, Uchida K, Grange DK, Singh GK, Nichols CG. Electrophysiologic consequences of KATP gain of function in the heart: conduction abnormalities in Cantu syndrome. Heart Rhythm 2015;12:2316-24.
20. Baczkó I, Husti Z, Lang V, Leprán I, Light PE. Sarcolemmal KATP channel modulators and cardiac arrhythmias. Curr Med Chem 2011;18:3640-61.
21. Gao J, McClenaghan C, Matreyek KA, Grange DK, Nichols CG. Rapid characterization of the functional and pharmacological consequences of cantú syndrome KATP channel mutations in intact cells. J Pharmacol Exp Ther 2023;386:298-309.
22. Cooper PE, Sala-Rabanal M, Lee SJ, Nichols CG. Differential mechanisms of Cantú syndrome-associated gain of function mutations in the ABCC9 (SUR2) subunit of the KATP channel. J Gen Physiol 2015;146:527-40.
23. McClenaghan C, Nichols CG. Kir6.1 and SUR2B in Cantú syndrome. Am J Physiol Cell Physiol 2022;323:C920-35.
24. Wrzosek A, Augustynek B, Żochowska M, Szewczyk A. Mitochondrial potassium channels as druggable targets. Biomolecules 2020;10:1200.
26. Garlid KD, Paucek P, Yarov-Yarovoy V, et al. Cardioprotective effect of diazoxide and its interaction with mitochondrial ATP-sensitive K+ channels. Possible mechanism of cardioprotection. Circ Res 1997;81:1072-82.
28. Shake JG, Peck EA, Marban E, et al. Pharmacologically induced preconditioning with diazoxide: a novel approach to brain protection. Ann Thorac Surg 2001;72:1849-54.
29. Caparrelli DJ, Cattaneo Ii SM, Bethea BT, et al. Pharmacological preconditioning ameliorates neurological injury in a model of spinal cord ischemia. Ann Thorac Surg 2002;74:838-45.
31. O’Rourke B. Evidence for mitochondrial K+ channels and their role in cardioprotection. Circ Res 2004;94:420-32.
32. Garlid KD. Opening mitochondrial KATP in the heart - what happens, and what does not happen. Basic Res Cardiol 2000;95:275-9.
33. Garlid KD, Dos Santos P, Xie ZJ, Costa AD, Paucek P. Mitochondrial potassium transport: the role of the mitochondrial ATP-sensitive K+ channel in cardiac function and cardioprotection. Biochim Biophys Acta 2003;1606:1-21.
34. Hu H, Sato T, Seharaseyon J, et al. Pharmacological and histochemical distinctions between molecularly defined sarcolemmal KATP channels and native cardiac mitochondrial KATP channels. Mol Pharmacol 1999;55:1000-5.
35. Gross GJ, Fryer RM. Sarcolemmal versus mitochondrial ATP-sensitive K+ channels and myocardial preconditioning. Circ Res 1999;84:973-9.
36. Kulawiak B, Szewczyk A. Current challenges of mitochondrial potassium channel research. Front Physiol 2022;13:907015.
37. Tseng GN, Hoffman BF. Actions of pinacidil on membrane currents in canine ventricular myocytes and their modulation by intracellular ATP and cAMP. Pflugers Arch 1990;415:414-24.
38. Inagaki N, Gonoi T, Clement JP, et al. A family of sulfonylurea receptors determines the pharmacological properties of ATP-sensitive K+ channels. Neuron 1996;16:1011-7.
39. Seino S. ATP-sensitive potassium channels: a model of heteromultimeric potassium channel/receptor assemblies. Annu Rev Physiol 1999;61:337-62.
40. Shyng SL, Nichols CG. Octameric stoichiometry of the KATP channel complex. J Gen Physiol 1997;110:655-64.
41. Sellitto AD, Maffit SK, Al-Dadah AS, et al. Diazoxide maintenance of myocyte volume and contractility during stress: evidence for a non-sarcolemmal KATP channel location. J Thorac Cardiovasc Surg 2010;140:1153-9.
42. Garlid KD, Halestrap AP. The mitochondrial KATP channel - fact or fiction? J Mol Cell Cardiol 2012;52:578-83.
43. Hu X, Xu X, Huang Y, et al. Disruption of sarcolemmal ATP-sensitive potassium channel activity impairs the cardiac response to systolic overload. Circ Res 2008;103:1009-17.
44. Flagg TP, Kurata HT, Masia R, et al. Differential structure of atrial and ventricular KATP: atrial KATP channels require SUR1. Circ Res 2008;103:1458-65.
45. Paggio A, Checchetto V, Campo A, et al. Identification of an ATP-sensitive potassium channel in mitochondria. Nature 2019;572:609-13.
46. Garlid KD, Paucek P. Mitochondrial potassium transport: the K+ cycle. Biochim Biophys Acta 2003;1606:23-41.
47. Kravenska Y, Checchetto V, Szabo I. Routes for potassium ions across mitochondrial membranes: a biophysical point of view with special focus on the ATP-sensitive K+ channel. Biomolecules 2021;11:1172.
48. Flagg TP, Enkvetchakul D, Koster JC, Nichols CG. Muscle KATP channels: recent insights to energy sensing and myoprotection. Physiol Rev 2010;90:799-829.
50. Rubin AA, Roth FE, Taylor RM, Rosenkilde H. Pharmacology of diazoxide, an antihypertensive, nondiuretic benzothiadiazine. J Pharmacol Exp Ther 1962;136:344-52. Avaliable from: https://jpet.aspetjournals.org/content/136/3/344.short [Last accessed on 26 Dec 2023].
52. Kumar GK, Dastoor FC, Rodriguez Robayo J, Razzaque MA. Side effects of diazoxide. JAMA 1976;235:275-6.
53. Komatsu Y, Nakamura A, Takihata M, et al. Safety and tolerability of diazoxide in Japanese patients with hyperinsulinemic hypoglycemia. Endocr J 2016;63:311-4.
54. Gray KD, Dudash K, Escobar C, et al. Best Pharmaceuticals for Children Act-Pediatric Trials Network Steering Committee. Prevalence and safety of diazoxide in the neonatal intensive care unit. J Perinatol 2018;38:1496-502.
55. Brito PC, Lopes V, Antunes E, Alves M, Gonçalves I, Matos AC. Hypoglycemia in a non-diabetic patient and the side effects of diazoxide use. Cureus 2023;15:e36804.
56. Quayle JM, Nelson MT, Standen NB. ATP-sensitive and inwardly rectifying potassium channels in smooth muscle. Physiol Rev 1997;77:1165-232.
57. Aziz Q, Li Y, Anderson N, Ojake L, Tsisanova E, Tinker A. Molecular and functional characterization of the endothelial ATP-sensitive potassium channel. J Biol Chem 2017;292:17587-97.
58. Wrzosek A, Gałecka S, Żochowska M, Olszewska A, Kulawiak B. Alternative targets for modulators of mitochondrial potassium channels. Molecules 2022;27:299.
59. Wang J, Papanicolaou K, Tryon R, et al. Kir1.1 and SUR1 are not implicated as subunits of an adenosine triphosphate-sensitive potassium channel involved in diazoxide cardioprotection. JTCVS Open 2023;15:231-41.
60. Anastacio MM, Kanter EM, Makepeace C, et al. Cardioprotective mechanism of diazoxide involves the inhibition of succinate dehydrogenase. Ann Thorac Surg 2013;95:2042-50.
61. Prasad SM, Al-Dadah AS, Byrd GD, et al. Role of the sarcolemmal adenosine triphosphate-sensitive potassium channel in hyperkalemic cardioplegia-induced myocyte swelling and reduced contractility. Ann Thorac Surg 2006;81:148-53.
62. Henn MC, Janjua MB, Kanter EM, et al. Adenosine triphosphate-sensitive potassium channel Kir subunits implicated in cardioprotection by diazoxide. J Am Heart Assoc 2015;4:e002016.
63. Henn MC, Janjua MB, Zhang H, et al. Diazoxide cardioprotection is independent of adenosine triphosphate-sensitive potassium channel Kir6.1 subunit in response to stress. J Am Coll Surg 2015;221:319-25.
64. Sellitto AD, Al-Dadah AS, Schuessler RB, Nichols CG, Lawton JS. An open sarcolemmal adenosine triphosphate-sensitive potassium channel is necessary for detrimental myocyte swelling secondary to stress. Circulation 2011;124:S70-4.
65. Henn MC, Janjua MB, Zhang H, et al. Increased tolerance to stress in cardiac expressed gain-of-function of adenosine triphosphate-sensitive potassium channel subunit Kir6.1. J Surg Res 2016;206:460-5.
66. Wang J, Suarez-Pierre A, Dong J, Lawton JS. Abstract 17206: implication of potassium inward rectifying (Kir1.1) Channel component in cardioprotective mechanism of adenosine triphosphate-sensitive potassium channel opener diazoxide. Circulation 2019;140:A17206. Avaliable from: https://www.ahajournals.org/doi/abs/10.1161/circ.140.suppl_1.17206 [Last accessed on 18 Jan 2024].
67. Rotko D, Kunz WS, Szewczyk A, Kulawiak B. Signaling pathways targeting mitochondrial potassium channels. Int J Biochem Cell Biol 2020;125:105792.
68. Auchampach JA, Grover GJ, Gross GJ. Blockade of ischaemic preconditioning in dogs by the novel ATP dependent potassium channel antagonist sodium 5-hydroxydecanoate downloaded from. Cardiovasc Res 1992;26:1054-62.
69. Shigematsu S, Sato T, Abe T, Saikawa T, Sakata T, Arita M. Pharmacological evidence for the persistent activation of ATP-sensitive K+ channels in early phase of reperfusion and its protective role against myocardial stunning. Circulation 1995;92:2266-75.
70. Galifianes M, Shattock MJ, Hearse DJ. Effects of potassium channel modulation during global ischaemia in isolated rat heart with and without cardioplegia. Cardiovasc Res 1992;26:1063-8.
71. Grover GJ, Sleph PG, Parham CS. Nicorandil Improves postischemic contractile function independently of direct myocardial effects. J Cardiovasc Pharmacol 1990;15:698-705.
72. Pignac J, Bourgouin J, Dumont L. Cold cardioplegia and the K+ channel modulator aprikalim (RP 52891): improved cardioprotection in isolated ischemic rabbit hearts. Can J Physiol Pharmacol 1994;72:126-32.
73. Janjua MB, Makepeace CM, Anastacio MM, Schuessler RB, Nichols CG, Lawton JS. Cardioprotective benefits of adenosine triphosphate-sensitive potassium channel opener diazoxide are lost with administration after the onset of stress in mouse and human myocytes. J Am Coll Surg 2014;219:803-13.
74. Tsuchida A, Miura T, Miki T, et al. Critical timing of mitochondrial K ATP channel opening for enhancement of myocardial tolerance against infarction. Basic Res Cardiol 2001;96:446-53.
75. Kleinbongard P, Lieder H, Skyschally A, Heusch G. Diazoxide is a powerful cardioprotectant but is not feasible in a realistic infarct scenario. Front Cardiovasc Med 2023;10:1173462.
76. Deja MA, Golba KS, Malinowski M, et al. Diazoxide provides maximal KATP channels independent protection if present throughout hypoxia. Ann Thorac Surg 2006;81:1408-16.
77. Mizutani S, Prasad SM, Sellitto AD, Schuessler RB, Damiano RJ Jr, Lawton JS. Myocyte volume and function in response to osmotic stress: observations in the presence of an adenosine triphosphate-sensitive potassium channel opener. Circulation 2005;112:I219-23.
78. Breisblatt WM, Stein KL, Wolfe CJ, et al. Acute myocardial dysfunction and recovery: a common occurrence after coronary bypass surgery. J Am Coll Cardiol 1990;15:1261-9.
79. Velez A, Sebestyen K, Lawton J. A novel, clinically useful definition of myocardial stunning after cardiac surgery. J Am Coll Cardiol 2022;79:1042.
80. Heyndrickx GR, Millard RW, Mcritchie RJ, Maroko PR, Vatner SF. Regional myocardial functional and electrophysiological alterations after brief coronary artery occlusion in conscious dogs. J Clin Invest 1975;56:978-85.
81. Torregroza C, Raupach A, Feige K, Weber NC, Hollmann MW, Huhn R. Perioperative cardioprotection: general mechanisms and pharmacological approaches. Anesth Analg 2020;131:1765-80.
82. Mizutani S, Al-Dadah AS, Bloch JB, et al. Hyperkalemic cardioplegia-induced myocyte swelling and contractile dysfunction: prevention by diazoxide. Ann Thorac Surg 2006;81:154-9.
83. Murry CE, Jennings RB, Reimer KA. Preconditioning with ischemia: a delay of lethal cell injury in ischemic myocardium. Circulation 1986;74:1124-36.
84. Gross GJ, Auchampach JA. Blockade of ATP-sensitive potassium channels prevents myocardial preconditioning in dogs. Circ Res 1992;70:223-33.
85. Ishida T, Yarimizu K, Gute DC, Korthuis RJ. Mechanisms of ischemic preconditioning. Shock 1994;8:86-94.
86. Wakahara N, Katoh H, Yaguchi Y, et al. Difference in the cardioprotective mechanisms between ischemic preconditioning and pharmacological preconditioning by diazoxide in rat hearts. Circ J 2004;68:156-62.
87. Sutherland FJ, Shattock MJ, Baker KE, Hearse DJ. Mouse isolated perfused heart: characteristics and cautions. Clin Exp Pharmacol Physiol 2003;30:67-78.
88. Motayagheni N. Modified Langendorff technique for mouse heart cannulation: improved heart quality and decreased risk of ischemia. MethodsX 2017;4:508-12.
89. Watanabe M, Okada T. Langendorff perfusion method as an ex vivo model to evaluate heart function in rats. In: Ishikawa K, editor. Experimental models of cardiovascular diseases. New York: Springer; 2018. pp. 107-16.
90. Lawton JS, Hsia PW, Damiano RJ. The adenosine-triphosphate-sensitive potassium-channel opener pinacidil is effective in blood cardioplegia. Ann Thorac Surg 1998;66:768-73.
91. Ledingham S, Braimbridge M, Hearse D. The St. Thomas’ Hospital cardioplegic solution: a comparison of the efficacy of two formulations. J Thorac Cardiovasc Surg 1987;93:240-6.
92. Lawton JS, Sepic JD, Allen CT, Hsia PW, Damiano RJ. Myocardial protection with potassium-channel openers is as effective as St. Thomas’ solution in the rabbit heart. Ann Thorac Surg 1996;62:31-9.
93. Lawton JS, Harrington GC, Allen CT, Hsia PW, Damiano RJ. Myocardial protection with pinacidil cardioplegia in the blood-perfused heart. Ann Thorac Surg 1996;61:1680-8.
94. Lawton JS, Hsia PW, Allen CT, Damiano RJ. Myocardial protection in the acutely injured heart: hyperpolarizing versus depolarizing hypothermic cardioplegia. J Thorac Cardiovasc Surg 1997;113:567-75.
95. Garlid KD, Paucek P, Yarov-Yarovoy V, Sun X, Schindler PA. The mitochondrial KATP channel as a receptor for potassium channel openers. J Biol Chem 1996;271:8796-9.
96. Andersson KE. Clinical pharmacology of potassium channel openers. Pharmacol Toxicol 1992;70:244-54.
97. Faivre JF, Findlay I. Effects of tolbutamide, glibenclamide and diazgxide upon action potentials recorded from rat ventricular muscle. Biochim Biophys Acta Bioenerg 1989;984:1-5.
98. Lascano EC, Negroni JA, Del Valle HF. Ischemic shortening of action potential duration as a result of KATP channel opening attenuates myocardial stunning by reducing calcium influx. Mol Cell Biochem 2002;236:53-61.
99. Inoue I, Nagase H, Kishi K, Higuti T. ATP-sensitive K+ channel in the mitochondrial inner membrane. Nature 1991;352:244-7.
100. Rousou AJ, Ericsson M, Federman M, Levitsky S, Mccully JD. Opening of mitochondrial KATP channels enhances cardioprotection through the modulation of mitochondrial matrix volume, calcium accumulation, and respiration. Am J Physiol Heart Circ Physiol 2004;287:1967-76.
101. Nakai Y, Horimoto H, Mieno S, Sasaki S. Mitochondrial ATP-sensitive potassium channel plays a dominant role in ischemic preconditioning of rabbit heart. Eur Surg Res 2001;33:57-63.
102. Coetzee WA. Multiplicity of effectors of the cardioprotective agent, diazoxide. Pharmacol Ther 2013;140:167-75.
103. Anastacio MM, Kanter EM, Makepeace CM, et al. Relationship between mitochondrial matrix volume and cellular volume in response to stress and the role of ATP-sensitive potassium channel. Circulation 2013;128:S130-5.
104. Murata M, Akao M, O’Rourke B, Marbán E. Mitochondrial ATP-sensitive potassium channels attenuate matrix Ca2+ overload during simulated ischemia and reperfusion: possible mechanism of cardioprotection. Circ Res 2001;89:891-8.
105. Steenbergen C, Hill ML, Jennings RB. Volume regulation and plasma membrane injury in aerobic, anaerobic, and ischemic myocardium in vitro. Effects of osmotic cell swelling on plasma membrane integrity. Circ Res 1985;57:864-75.
106. Al-Dadah AS, Voeller RK, Schuessler RB, Damiano RJ Jr, Lawton JS. Maintenance of myocyte volume homeostasis during stress by diazoxide is cardioprotective. Ann Thorac Surg 2007;84:857-62.
107. Maffit SK, Sellitto AD, Al-Dadah AS, Schuessler RB, Damiano RJ Jr, Lawton JS. Diazoxide maintains human myocyte volume homeostasis during stress. J Am Heart Assoc 2012;1:e000778.
108. Zhang HX, Akrouh A, Kurata HT, Remedi MS, Lawton JS, Nichols CG. HMR 1098 is not an SUR isotype specific inhibitor of heterologous or sarcolemmal KATP channels. J Mol Cell Cardiol 2011;50:552-60.
109. Suzuki M, Saito T, Sato T, et al. Cardioprotective effect of diazoxide is mediated by activation of sarcolemmal but not mitochondrial ATP-sensitive potassium channels in mice. Circulation 2003;107:682-5.
110. Wojtovich AP, Brookes PS. The endogenous mitochondrial complex II inhibitor malonate regulates mitochondrial ATP sensitive potassium channels: implications for ischemic preconditioning. Biochim Biophys Acta 2008;1777:882-9.
111. Ardehali H, Chen Z, Ko Y, Mejía-Alvarez R, Marbá E. Multiprotein complex containing succinate dehydrogenase confers mitochondrial ATP-sensitive K+ channel activity. Proc Natl Acad Sci 2004;101:11880-5.
112. Krenz M, Oldenburg O, Wimpee H, et al. Opening of ATP-sensitive potassium channels causes generation of free radicals in vascular smooth muscle cells. Basic Res Cardiol 2002;97:365-73.
113. Zeng WZ, Liou HH, Murali Krishna U, Falck JR, Huang CL. Structural determinants and specificities for ROMK1-phosphoinositide interaction. Am J Physiol-Renal 2002;282:826-34.
114. Forbes RA, Steenbergen C, Murphy E. Diazoxide-induced cardioprotection requires signaling through a redox-sensitive mechanism. Circ Res 2001;88:802-9.
115. Carroll R, Gant VA, Yellon DM. Mitochondrial KATP channel opening protects a human atrial-derived cell line by a mechanism involving free radical generation. Cardiovasc Res 2001;51:691-700.
116. Oldenburg O, Yang XM, Krieg T, et al. P1075 opens mitochondrial KATP channels and generates reactive oxygen species resulting in cardioprotection of rabbit hearts. J Mol Cell Cardiol 2003;35:1035-42.
117. Garlid AO, Jaburek M, Jacobs JP, Garlid KD. Mitochondrial reactive oxygen species: which ROS signals cardioprotection? J Physiol Heart Circ Physiol 2013;305:960-8.
118. Dröse S, Brandt U, Hanley PJ. K+-independent actions of diazoxide question the role of inner membrane KATP channels in mitochondrial cytoprotective signaling. J Biol Chem 2006;281:23733-9.
119. Hanley PJ, Mickel M, Löffler M, Brandt U, Daut J. KATP channel-independent targets of diazoxide and 5-hydroxydecanoate in the heart. J Physiol 2002;542:735-41.
120. Dzeja PP, Bast P, Ozcan C, et al. Targeting nucleotide-requiring enzymes: implications for diazoxide-induced cardioprotection. Am J Physiol Heart Circ Physiol 2003;284:1048-56.
121. Ockaili RA, Bhargava P, Kukreja RC. Chemical preconditioning with 3-nitropropionic acid in hearts: role of mitochondrial KATP channel. J Physiol Heart Circ Physiol 2001;280:2406-11.
122. Busija DW, Katakam P, Rajapakse NC, et al. Effects of ATP-sensitive potassium channel activators diazoxide and BMS-191095 on membrane potential and reactive oxygen species production in isolated piglet mitochondria. Brain Res Bull 2005;66:85-90.
123. Chouchani ET, Pell VR, Gaude E, et al. Ischaemic accumulation of succinate controls reperfusion injury through mitochondrial ROS. Nature 2014;515:431-5.
124. Anastacio MM, Kanter EM, Keith AD, Schuessler RB, Nichols CG, Lawton JS. Inhibition of succinate dehydrogenase by diazoxide is independent of the ATP-sensitive potassium channel subunit sulfonylurea type 1 receptor. J Am Coll Surg 2013;216:1144-9.
125. Eaton M, Hernandez LA, Schaefer S. Ischemic preconditioning and diazoxide limit mitochondrial Ca2+ overload during ischemia/reperfusion: role of reactive oxygen species. Exp Clin Cardiol 2005;10:96-103.
126. Ahmad T, Wang J, Velez AK, et al. Cardioprotective mechanisms of mitochondria-targeted S-nitrosating agent and adenosine triphosphate-sensitive potassium channel opener are mutually exclusive. JTCVS Open 2021;8:338-54.
127. Uchiyama Y, Otani H, Okada T, et al. Integrated pharmacological preconditioning in combination with adenosine, a mitochondrial KATP channel opener and a nitric oxide donor. J Thorac Cardiovasc Surg 2003;126:148-59.
128. Kim MY, Kim MJ, Yoon IS, et al. Diazoxide acts more as a PKC-epsilon activator, and indirectly activates the mitochondrial KATP channel conferring cardioprotection against hypoxic injury. Br J Pharmacol 2006;149:1059-70.
129. Akao M, Ohler A, O’rourke B, Marbán E. Mitochondrial ATP-sensitive potassium channels inhibit apoptosis induced by oxidative stress in cardiac cells. Circ Res 2001;88:1267-75.
130. Yonemochi H, Ichinose M, Anan F, et al. Diazoxide-induced cardioprotection via DeltaPsim loss depending on timing of application. Life Sci 2006;79:1906-12.
131. Mccully JD, Wakiyama H, Cowan DB, Federman M, Parker RA, Levitsky S. Diazoxide amelioration of myocardial injury and mitochondrial damage during cardiac surgery. Ann Thorac Surg 2002;74:2138-46.
132. Maslov LN, Popov SV, Naryzhnaya NV, et al. KATP channels are regulators of programmed cell death and targets for the creation of novel drugs against ischemia/reperfusion cardiac injury. Fundam Clin Pharmacol 2023;37:1020-49.
133. Davies JE, Digerness SB, Killingsworth CR, et al. Multiple treatment approach to limit cardiac ischemia-reperfusion injury. Ann Thorac Surg 2005;80:1408-16.
134. Makepeace CM, Suarez-Pierre A, Kanter EM, Schuessler RB, Nichols CG, Lawton JS. Superior diastolic function with KATP channel opener diazoxide in a novel mouse Langendorff model. J Surg Res 2018;227:186-93.
135. Suarez-Pierre A, Lui C, Zhou X, et al. Diazoxide preserves myocardial function in a swine model of hypothermic cardioplegic arrest and prolonged global ischemia. J Thorac Cardiovasc Surg 2022;163:e385-400.
136. Velez AK, Etchill E, Giuliano K, et al. ATP - sensitive potassium channel opener diazoxide reduces myocardial stunning in a porcine regional with subsequent global ischemia model. J Am Heart Assoc 2022;11:e026304.
137. Wang X, Wei M, Kuukasjärvi P, et al. Novel pharmacological preconditioning with diazoxide attenuates myocardial stunning in coronary artery bypass grafting. Eur J Cardiothorac Surg 2003;24:967-73.
138. Deja MA, Malinowski M, Gołba KS, et al. Diazoxide protects myocardial mitochondria, metabolism, and function during cardiac surgery: a double-blind randomized feasibility study of diazoxide-supplemented cardioplegia. J Thorac Cardiovasc Surg 2009;137:997-1004.
139. Ziganshin BA, Elefteriades JA. Deep hypothermic circulatory arrest. Ann Cardiothorac Surg 2013;2:303-15.
140. Wang X, Yang F, Zhu J, Liu Y, Sun L, Hou X. Aortic arch surgery with hypothermic circulatory arrest and unilateral antegrade cerebral perfusion: perioperative outcomes. J Thorac Cardiovasc Surg 2020;159:374-87.e4.
141. Bergeron EJ, Mosca MS, Aftab M, Justison G, Reece TB. Neuroprotection strategies in aortic surgery. Cardiol Clin 2017;35:453-65.
142. Giuliano K, Etchill E, Velez AK, et al. Ketamine mitigates neurobehavioral deficits in a canine model of hypothermic circulatory arrest. Semin Thorac Cardiovasc Surg 2023;35:251-8.
143. Honrath B, Krabbendam IE, Culmsee C, Dolga AM. Small conductance Ca2+-activated K+ channels in the plasma membrane, mitochondria and the ER: pharmacology and implications in neuronal diseases. Neurochem Int 2017;109:13-23.
144. Debska G, May R, Kicinska A, Szewczyk A, Elger CE, Kunz WS. Potassium channel openers depolarize hippocampal mitochondria. Brain Res 2001;892:42-50.
145. De Arriba S, Franke H, Pissarek M, Nieber K, Illes P. Neuroprotection by ATP-dependent potassium channels in rat neocortical brain slices during hypoxia. Neurosci Lett 1999;273:13-6.
146. Domoki F, Perciaccante JV, Veltkamp R, Bari F, Busija DW. Mitochondrial Potassium channel opener diazoxide preserves neuronal-vascular function after cerebral ischemia in newborn pigs. Stroke 1999;30:2713-9.
147. Barreiro CJ, Williams JA, Fitton TP, et al. Noninvasive assessment of brain injury in a canine model of hypothermic circulatory arrest using magnetic resonance spectroscopy. Ann Thorac Surg 2006;81:1593-8.
148. Teshima Y, Akao M, Li RA, et al. Mitochondrial ATP-sensitive potassium channel activation protects cerebellar granule neurons from apoptosis induced by oxidative stress. Stroke 2003;34:1796-802.
149. Fatemi A, Wilson MA, Johnston MV. Hypoxic-ischemic encephalopathy in the term infant. Clin Perinatol 2009;36:835-58, vii.
150. Yamanaka K, Eldeiry M, Aftab M, et al. Pretreatment with diazoxide attenuates spinal cord ischemia-reperfusion injury through signaling transducer and activator of transcription 3 pathway. Ann Thorac Surg 2019;107:733-9.
151. Yamanaka K, Eldeiry M, Aftab M, et al. Synergistic reduction of apoptosis with diazoxide and erythropoietin in spinal cord ischemic injury. Ann Thorac Surg 2018;106:1751-8.
152. Yamanaka K, Eldeiry M, Aftab M, et al. Synergetic induction of NGF with diazoxide and erythropoietin attenuates spinal cord ischemic injury. J Surg Res 2019;233:124-31.
Cite This Article
Export citation file: BibTeX | RIS
OAE Style
Bradshaw AB, Lawton JS. Exploitation of KATP channels for cardiac surgery. Vessel Plus 2023;7:35. http://dx.doi.org/10.20517/2574-1209.2023.121
AMA Style
Bradshaw AB, Lawton JS. Exploitation of KATP channels for cardiac surgery. Vessel Plus. 2023; 7: 35. http://dx.doi.org/10.20517/2574-1209.2023.121
Chicago/Turabian Style
Bradshaw, AlleaBelle, Jennifer S. Lawton. 2023. "Exploitation of KATP channels for cardiac surgery" Vessel Plus. 7: 35. http://dx.doi.org/10.20517/2574-1209.2023.121
ACS Style
Bradshaw, AB.; Lawton JS. Exploitation of KATP channels for cardiac surgery. Vessel Plus. 2023, 7, 35. http://dx.doi.org/10.20517/2574-1209.2023.121
About This Article
Special Issue
Copyright
Data & Comments
Data
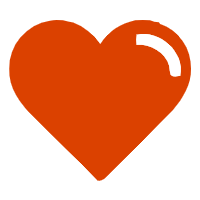

Comments
Comments must be written in English. Spam, offensive content, impersonation, and private information will not be permitted. If any comment is reported and identified as inappropriate content by OAE staff, the comment will be removed without notice. If you have any queries or need any help, please contact us at support@oaepublish.com.